September 17, 2019 feature
Bioengineering organ-specific tissues with high cellular density and embedded vascular channels
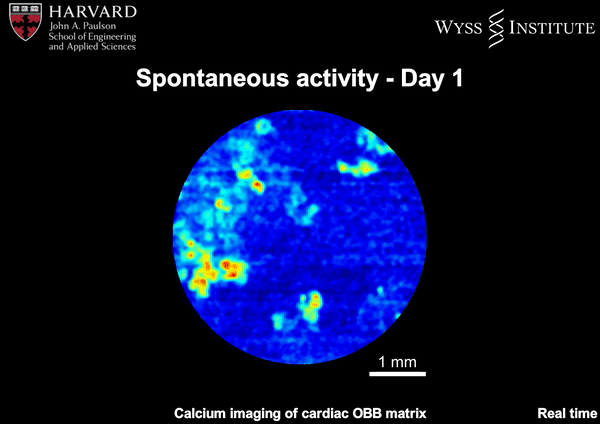
Bioengineers study the development of organ-specific tissues in the lab for therapeutic applications. However, the process is highly challenging, since it requires the fabrication and maintenance of dense cellular constructs composed of approximately 108 cell/mL. Research teams have used organ building blocks (OBBs) composed of patient-specific-induced pluripotent stem cell (iPSC)-derived organoids as a pathway to achieve the requisite cell density, microarchitecture and tissue function. However, OBBs hitherto remain to be assembled into 3-D tissue constructs. In a recent report, Mark A. Skylar-Scott and an interdisciplinary research team at the Wyss Institute for Biologically Inspired Engineering and the John A. Paulson School of Engineering and Applied Sciences at Harvard University, developed a new biomanufacturing method.
Instead of 3-D printing constructs to fill in with cells, the scientists assembled thousands of OBBs into living matrices with high cellular density, into which they introduced perfusable vascular channels using 3-D bioprinting. The OBB matrices exhibited the desired self-healing and viscoelastic behavior to convert sacrificial writing into functional tissue (SWIFT). As an example, they engineered a perfusable cardiac tissue to fuse and beat synchronously across a timeframe of seven days. The SWIFT biomanufacturing method allowed the rapid assembly of patient- and organ-specific tissues at therapeutic scales. The research work is now published in Science Advances.
Bioengineering whole organs for therapeutic applications is a daunting task since billions of cells are required for rapid organization into functional microarchitectures, with nutrient supplements via vascular channels. Recent advances in tissue engineering have led to the self-assembly of cerebral, kidney and cardiac organoids, with several characteristics similar to their in vivo organ counterparts. Scientists build such organoids by generating embryoid bodies (EBs) made of iPSCs (induced pluripotent stem cells) within microwells, cultured under static conditions to differentiate into 'mini organs' of interest. Such organs serve as ideal organ building blocks (OBBs) to biomanufacture tissues of interest with the desired cell density, character, microarchitecture and function.
Researchers can then introduce a perfusable network of vascular channels into the engineered living matrices using embedded 3-D printing techniques. For example, when research teams introduced a method known as sacrificial ink writing into cellular hydrogels and silicone matrices, the outcomes resulted in a 3-D network of interconnected channels. By building on this strategy, bioengineers proceeded to develop synthetic and biopolymer matrices with self-healing, viscoelastic responses for minimal complexity of the patterning protocols to form 3-D architectures. However, researchers had thus far only used this method to construct either acellular or spatially cellular matrices.
![Sacrificial writing into functional tissue (SWIFT). (A) Step-by-step illustration of the SWIFT process. (B) (i) Large-scale microwell culture of approximately (ii) 2.5 ml of EB-based OBBs, compacted to form an (iii) OBB tissue matrix composed of approximately half a billion cells. Scale bar, 300 μm (i). Scale bar, is 200 μm (iii). (C) Time-lapse of sacrificial ink (red) writing via embedded 3D printing within an EB matrix observed from beneath the reservoir. (D) Front view of a vertical line of sacrificial ink printed within an EB matrix. Scale bars, 1 mm (C and D). (E) Examples of the SWIFT process for different OBB-based matrices composed of the following: (i) EBs, (ii) cerebral organoids, and (iii) cardiac spheroids. Row 1: Individual OBBs with characteristic markers. Rows 2 and 3: Cross sections [as indicated in (D) by the dashed line] of immunostained slices and bright-field images, respectively, of the OBB types. Scale bars, 50 μm (top row) and 500 μm (middle and bottom rows). (F) Generation of a helical (vascular) feature in an EB matrix via SWIFT: (i) CAD representation of the system and (ii) corresponding image of sacrificial ink writing within an EB matrix, and (iii) image sequence acquired during embedded 3D printing of a sacrificial ink (left), sacrificial ink evacuation upon incubation (middle), and tissue perfusion using media (dyed blue) through the printed helical vascular channels. Credit: Science Advances, doi: 10.1126/sciadv.aaw2459 Bioengineering organ-specific tissues with high cellular density and embedded vascular channels.](https://scx1.b-cdn.net/csz/news/800a/2019/bioengineeri.jpg)
In the present work, therefore, Skylar-Scott et al. developed a biomanufacturing protocol that relied on sacrificial writing into functional tissue (SWIFT) composed of a living OBB matrix to generate organ-specific tissues with high cellular density, maturation and desired functionality.
- They first engineered significant magnitudes of iPSC-derived OBBs in the form of EBs (embryoid bodies), organoids or multicellular spheroids.
- They followed this step by placing the OBBs into a mold to form a living OBB matrix.
- Thereafter, they rapidly patterned a sacrificial ink in the matrix using embedded 3-D printing, which, upon removal, produced perfusable (enabling nutrient flow) single or branching conduit channels.
The scientists then demonstrated the function and maturation of the engineered bulk vascularized tissue during long-term perfusion studies.
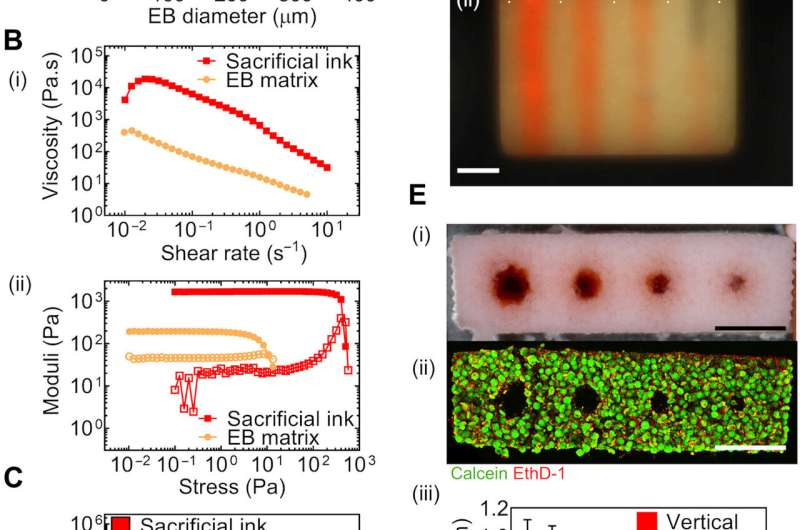
The research team grew the iPSCs in adherent culture and transferred them into large-scale microwell arrays to form a large volume of EBs and OBBs for the SWIFT protocol. The composite extracellular matrix (ECM) solution contained collagen and Matrigel as an ideal solution to build perfusable vascular channels due to its fluid-like behaviour at low temperatures, which also facilitated casting and embedded printing for sacrificial ink removal and tissue perfusion. They crushed the OBB-ECM slurry using centrifugation and produced a living tissue matrix containing nearly half a billion cells at a density approximating 200 million cells/mL. This provided sufficient support for embedded 3-D printing of a sacrificial gelatin ink to build a template for the vascular channel of interest.
The SWIFT biomanufacturing method required the self-healing character of OBB-based matrices, followed by matrix stiffening to remove the ink. The pronounced increase in the tissue matrix stiffness due to gelation at 37 degrees C, allowed the scientists to retain the structural integrity of the construct, while removing the sacrificial ink.
Due to its self-healing behavior, the scientists deposited the living tissue matrix without crevasses or defects using a translating nozzle. The team printed patent vascular channels to generate three distinct matrices to build (1) compacted EBs, (2) cerebral organoids (after differentiating for 21 days) and (3) beating cardiac spheroids based on iPSC-derived cardiomyocytes mixed with primary cardiac fibroblasts. As a result of its free-form nature, they used SWIFT to pattern nearly arbitrary vascular networks and they tailored the tissue matrix composition and ink rheology to develop a system to print vascular channels. As proof-of-concept, they printed an ink containing fluorescent protein-expressing EBs into a tissue matrix in a helical pattern.
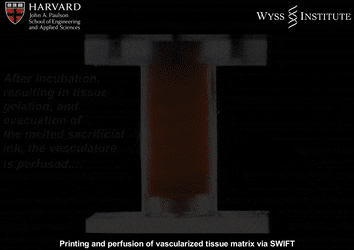
To investigate cell viability, the research team manufactured a perfusable EB-based tissue in a perfusion chamber containing circulating oxygenated media. In parallel, they used a non-perfusable EB-based tissue as a control without channels. The control tissue developed a necrotic core within 12 hours of culture with viable and metabolically active cells. In contrast, luminal perfusion of the EB-based tissue improved cell viability throughout the bulk tissue.
To scale up the biomanufacturing process, the team created a custom-shaped mold with an inlet and outlet for perfusion and added the EB-ECM slurry developed in the lab, with more than 40,000 EBs and a total of 0.5 billion cells. They printed a branched hierarchical channel network to distribute flow and maintain a constant wall shear stress throughout the tissue matrix using human umbilical vein endothelial cells (HUVECs) to form endothelial cell-lined channels. Such cells are typically found lining the inner wall of blood vessels and can promote a characteristic healing response around vascular implants in vivo. The team intends to further optimize the method to produce a more confluent endothelium within the printed vascular channels in the lab.
![Perfusable cardiac tissue fabricated by SWIFT. (A) Cardiac organoid differentiation protocol. (B) Cardiac troponin T and 4′,6-diamidino-2-phenylindole (DAPI) staining in a single cardiac OBB at day 9. Scale bar, 50 μm. (C) Cardiac troponin T, α-actinin, and DAPI staining in a single cardiac OBB at day 9. Scale bar, 10 μm. (D) Cardiac spheroid composition in iPSC-derived cardiac OBB. Cardiomyocytes (CM) are identified as cardiac troponin T-positive (cTnT+) and stromal-like cells (strom.) as cTnT−/Vimentin+. (E) Cellular density in compacted cardiac OBB tissue. (F) An image sequence showing the embedding, evacuation, and perfusion of branched vascular channels within a cardiac tissue matrix (tissue dimensions: top width, 6 mm; bottom width, 4.2 mm; depth, 4.2 mm; and height, 12 mm). Scale bars, 2 mm. (G) Viability staining of a SWIFT cardiac tissue (cross section) after 24 hours of perfusion. Scale bar, 500 μm. (H) cTnT, α-actinin, and DAPI staining in a SWIFT cardiac tissue after 8 days of perfusion that shows evidence of sarcomeric remodeling (arrowheads). Scale bar, 10 μm. (I) Vertical displacement of the anchoring flexible prongs due to spontaneous cardiomyocyte contraction showing increasing amplitude over time. On day 8, 2 mM calcium is added to the medium to increase cardiomyocyte contractility (“d8 + Ca”). (J) Comparison of anchor displacement pattern between spontaneous contraction and electrical pacing (1 and 2 Hz) of SWIFT cardiac tissues. (K) Spontaneous contraction pattern before and after administration of 10 μM isoproterenol. (L) Average contraction frequency under isoproterenol treatment. (M) Spontaneous contraction pattern before and after administration of 1 mM 1-heptanol. (N) Maximum peak-to-peak contraction amplitude under 1-heptanol treatment. (O) 3D CAD model of a normal human heart, including a segment of the left anterior descending (LAD) artery and a diagonal branch, downloaded from the National Institutes of Health 3D Print Exchange (additional septal and diagonal branches were added manually, pink). (P) A 1:2 scale polydimethylsiloxane mold is formed using the 3D computed tomography data, and the LAD artery together with diagonal and septal (arrowheads) branches are embedded into a septal-anterior wall wedge [yellow section in (O)] of the cardiac tissue matrix via SWIFT. Scale bar, 5 mm. Credit: Science Advances, doi: 10.1126/sciadv.aaw2459. Bioengineering organ-specific tissues with high cellular density and embedded vascular channels.](https://scx1.b-cdn.net/csz/news/800a/2019/2-bioengineeri.jpg)
Skylar-Scott et al. then created a functional, perfusable tissue containing human iPSC-derived cardiac OBBs. They adapted an existing protocol to form cardiomyocytes with high efficiency and generated beating cardiac OBBs containing large aggregates of cardiomyocytes (79 ± 6 percent) and stromal cells (19 ± 6 percent), with calcium waves propagating sporadically after a single day of perfusion. By day seven, the tissue constructs beat spontaneously and synchronously with rhythmic and rapid calcium wave propagation throughout the tissue.
As a final demonstration they printed an arterial vascular network geometry within a cardiac OBB matrix using patient-specific cardiac structural data, which they obtained from the National Institute of Health (NIH) 3-D Print Exchange. The researchers used a 3-D printed mold and replicated the myocardium at a 1:2 scale, filled it with compacted cardiac OBB matrix and used SWIFT to engineer the geometry of the left anterior descending (LAD) coronary artery.
In this way, Mark A. Skylar-Scott and colleagues developed the SWIFT biomanufacturing method to form dense cellular matrices composed of iPSC-derived OBBs to produce autologous tissues, engineered with patient-specific cells. The new method allowed them to create perfusable organ-specific tissues of specific volume and shape to scale. They used large volumes of perfusable OBB-based tissues for patterning with an embedded vascular channel network. The successful translation of these experimental tissue into the therapeutic domain will require additional optimization studies. The research team expect the new method to open new avenues to create personalized, organ specific tissues with embedded vascular channels for therapeutic applications.
More information: Mark A. Skylar-Scott et al. Biomanufacturing of organ-specific tissues with high cellular density and embedded vascular channels, Science Advances (2019). DOI: 10.1126/sciadv.aaw2459
Wolfram-Hubertus Zimmermann et al. Engineered heart tissue grafts improve systolic and diastolic function in infarcted rat hearts, Nature Medicine (2006). DOI: 10.1038/nm1394
Kelly R. Stevens et al. In situ expansion of engineered human liver tissue in a mouse model of chronic liver disease, Science Translational Medicine (2017). DOI: 10.1126/scitranslmed.aah5505
Journal information: Science Advances , Nature Medicine , Science Translational Medicine
© 2019 Science X Network